Microscopic imaging of a large number of biological samples is needed for a wide spectrum of applications in life sciences, including High Content Screening (HCS) protocols. However, conventional microscopy is limited by some technical limitations (movement speed of the stage, communication latency and device synchronization) that are not compatible with experiment protocols. This application note introduces the use of the Inscoper Imaging Solution to perform continuous motion imaging microscopy. The principle is to acquire images while the stage moves in continuous motion. With this new technique, researchers can monitor a large number of samples at very high speed with a preserved resolution. This commercial solution was first installed and tested at the National Institute of Standards and Technology (NIST; Gaithersburg, Maryland, USA).
Regular Tiling Imaging VS Continuous Motion Imaging
PRINCIPLE OF CONTINUOUS MOTION IMAGING
Automation in the field of microscopy has allowed researchers to easily image large samples in an automated manner using the motorized stage. For instance, it is now common in a single acquisition sequence to image large histological sections or various batches of live cells in culture in multi-well plates. Called High Content Imaging (HCI), this automation of acquisition protocols enables the development of high throughput techniques for the imaging of various samples, such as C.elegans (Maglioni et al, 2021), zebrafish(Lin et al, 2011) or organoids (Zhou et al, 2017). HCI can be used in many applications including fundamental research, target-based screening or cell-/animal-based phenotypic screening (Bray et al, 2016; Arias-Fuenzalida et al, 2019; Hoffman et al, 2021). Conventional automated microscopes use an incremental scanning routine to perform large imaging protocols (Figure 1A). Such an approach is composed of several steps including repeatedly positioning the object, adjusting the focus and capturing a segment of the stationary object.
However, this incompressible time limits temporal resolution when acquiring rapid biological phenomena such as protein synthesis, apoptosis, endocytosis, cell division, etc. Schenk et al, 2016 developed a new method of high-speed microscopy combining phase contrast and brightfield imaging to image large cell culture vessels. To dramatically increase the framerate, they proposed (i) to remove the stop of each acquisition and (ii) to image the samples during a continuous movement of the stage (Figure 1B). The system presented in the article is composed of a high-speed sCMOS camera, a pulsed LED, a motorized XY stage, a microscope with adapted objectives and a piezo Z-stage. All these elements were initially controlled by a C++ program developed in-house and by μManager software. The main challenge of continuous acquisition is to prevent the motion blur and ensure that samples remain in focus at all times.
Figure 1. Illustration of the mosaic steps with conventional and continuous motion imaging approaches
(A) In a conventional imaging protocol, the acquisition is sequential, alternating between stage movement and image capture. Each colored square represents a camera trig and each arrow highlights that the stage is moving. Space between the colored squares indicates the additional time for stage motion and camera triggering. (B) With continuous motion imaging, images are taken continuously during non-stop stage movement.
microscope integration
The Inscoper Imaging Solution (INSCOPER; Cesson-Sévigné, France) is a complete and optimized control and image acquisition solution for automated microscopes used in life science. It replaces the commercial acquisition software for Leica, Nikon, Evident (Olympus) or Zeiss microscopes, and can also equip home-built systems to make custom-made applications accessible to regular users.
The user-friendly graphical user interface allows full control of all motorized devices, configuration of multidimensional acquisition sequences (time, XYZ, channels, multi-positions, tiling) and image visualization and processing. The Inscoper core technology improves the temporal resolution of acquisitions by removing all software latency and optimizing the synchronization of the elements of the microscope.
INSCOPER has recently installed two systems to perform continuous motion acquisition (Figure 2).
Figure 2. Microscope setup for continuous motion microscopy with Inscoper Imaging Solution
The optimized setup used for continuous motion imaging microscopy is composed of a pulsed LED light source, a XY stage, a microscope, a high-speed camera and a piezo stage. All these devices are connected to the Inscoper device controller.
System #1: The optimized setup is composed of an AxioObserver Z1 (Zeiss, Oberkochen, Germany), a SCANplus motorized scanning stage (Märzhäuser, Wetzlar, Germany), an ORCA-fusion BT camera (C15440-20UP; Hamamatsu Photonics, Hamamatsu, Japan), a pulsed LED light source (LED100, Märzhäuser) and the Inscoper Imaging Solution (INSCOPER, Cesson-Sévigné, France).
System #2: The regular system is equipped with the same devices as above, but with a Ti2-E microscope and TI2-S-SE-E motorized stage (Nikon, Tokyo, Japan) instead of AxioObserver stand and Märzhäuser stage.
set up and acquisition
Acquisition protocols were manually created using the software interface. At the start of the acquisition, all settings are sent to the Inscoper device controller that will run the acquisition without any computer latency and with perfect synchronization between all devices. For continuous motion imaging, the exposure time has to be limited to a few microseconds using an intense LED flash to prevent motion blur with the best signal-to-noise ratio (SNR).
An automated calibration protocol has been implemented in the software to perfectly synchronize the LED flashes and camera trigger with the continuous movement of the stage. Users determine the time required for the stage to reach the desired motion speed. To do this, the acceleration and deceleration profiles of the stage are measured (Figure 3A). Once this fully automated calibration step is complete, continuous motion acquisitions can be customized and tailored to the experiment requirements (Figure 3B).
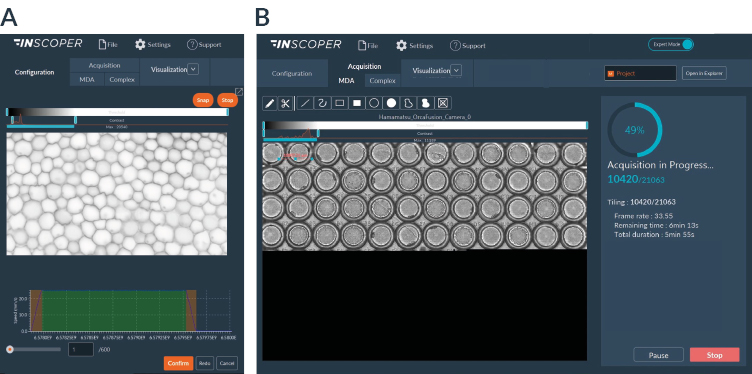
Figure 3. Inscoper software interface for continuous motion acquisition
(A) Continuous motion imaging requires perfect synchronization between the stage and the light source. The calibration step consists in determining in an automated way the acceleration and deceleration profile of the stage (orange part of the graph) while identifying the time required to obtain the desired displacement speed (green area of the graph). (B) Continuous motion acquisition allows real-time visualization of the sample with a preserved spatial and temporal resolution. Users can have an overview of their sample and interact in real time with stitched images.
This innovative imaging method allows researchers to easily image large biological samples and multiwell plate supports with a very high frame rate (Figure 4). For time lapse imaging this is a fundamental change to traditional imaging strategies and significantly improves the microscope’s spatial bandwidth product, i.e. the relationship between the temporal resolution of a microscope and the area that can be imaged within the time lapse interval. Researchers could explore a large panel of biological applications including the impact of drugs on cell survival, mitosis or the motion of single cells for example. The dynamic interactions of gene regulatory networks components could also potentially be examined with this technological advance (Plant, Halter, 2020).
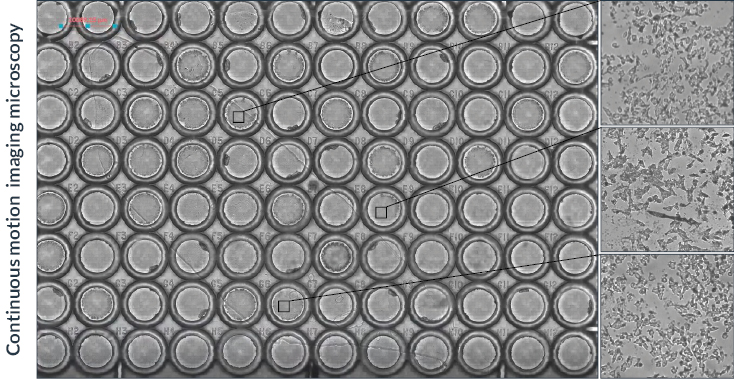
Figure 4. Continuous motion imaging for tiling acquisitions
Overview of the final assembled image obtained by continuous motion imaging. Live zooms can be performed during acquisition and in the Visualization tab to see in more detail each part of the 96-well plate, as shown on the right side of the panel.
results
Continuous motion acquisition enables the imaging of large samples at a very high frame rate. Different acquisitions of the same samples could be performed using the Inscoper solution without and with the continuous motion modality, and with the µManager software as a reference. The results are summarized
below (Table I).
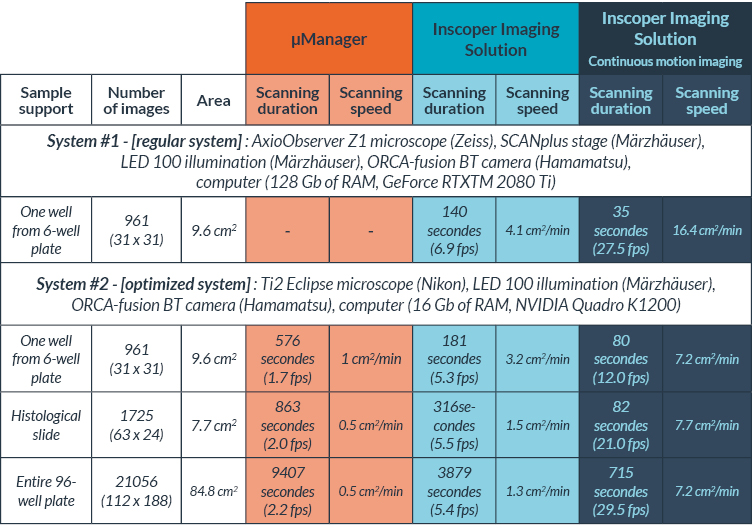
Table I. Comparison between µManager and Inscoper Imaging Solution for tiling acquisitions
Inscoper technology already ensures the highest speed for device control and image acquisition (about 3 times higher frame rate) compared to conventional microscopy software such as µManager or other commercial software. Continuous motion imaging increases acquisition speed even more by an additional factor of 3. The results were observed by comparing the acquisition of a histological slide (Figure 5A) and a whole 96-well plate (Figure 5B).
Figure 5. Very high-speed microscopy with a continuous motion imaging method
Comparison of the acquisition speed for imaging a histological slide (A) or 96-well plate (B) performed with µManager (orange), and Inscoper Imaging Solution regular modality (cyan), and continuous motion modality (blue night).
Imaging a 96-well plate (21,056 images) took 157 minutes and 47 seconds with µManager, 64 minutes and 39 seconds with Inscoper Imaging Solution and 11 minutes and 55 seconds with the continuous imaging modality. Continuous motion imaging with Inscoper has been around 13 times faster than µManager to image an entire 96-well plate (Figure 5B). The optimized system could not be equipped with µManager for compatibility issues, but the result would have been even more impressive.
Summary
Continuous motion imaging allows to dramatically increase the acquisition frame rate in brightfield imaging. Combined with the Inscoper Imaging Solution, this technique is even 3 times faster and can easily be implemented on most microscopes on the market. This innovative method is certainly very promising for High Content Imaging, but can also be interesting in other applications in biology or other fields.
Bibliography
-
- Appaduray MA, Masedunskas A, Bryce NS, Lucas CA, Warren SC, Timpson P, Stear JH, Gunning PW & Hardeman EC (2016) Recruitment Kinetics of Tropomyosin Tpm3.1 to Actin Filament Bundles in the Cytoskeleton Is Independent of Actin Filament Kinetics. PLOS One 11: 1–16
- Axelrod D, Koppel DE, Schlessinger J, Elson E & Webb WW (1976) Mobility measurement by analysis of fluorescence photobleaching recovery kinetics. Biophys J 16: 1055–1069
- Giese B, Au-Yeung C-K, Herrmann A, Diefenbach S, Haan C, Küster A, Wortmann SB, Roderburg C, Heinrich PC, Behrmann I, et al (2003) Long Term Association of the Cytokine Receptor gp130 and the Janus Kinase Jak1 Revealed by FRAP Analysis. J Biol Chem 278: 39205–39213
- Ishikawa-Ankerhold HC, Ankerhold R & Drummen GPC (2012) Advanced Fluorescence Microscopy Techniques—FRAP, FLIP, FLAP, FRET and FLIM. Molecules 17: 4047–4132
- Kang M, Day CA, DiBenedetto E & Kenworthy AK (2010) A Quantitative Approach to Analyze Binding Diffusion Kinetics by Confocal FRAP. Biophys J 99: 2737–2747
- Lippincott-Schwartz J, Snapp EL & Phair RD (2018) The Development and Enhancement of FRAP as a Key Tool for Investigating Protein Dynamics. Biophys J 115: 1146–1155
- Oakes SA (2020) Endoplasmic Reticulum Stress Signaling in Cancer Cells. Am J Pathol 190: 934–946
- Ozcan L & Tabas I (2012) Role of Endoplasmic Reticulum Stress in Metabolic Disease and Other Disorders. Annu Rev Med 63: 317–328
- Öztürk Z, O’Kane CJ & Pérez-Moreno JJ (2020) Axonal Endoplasmic Reticulum Dynamics and Its Roles in Neurodegeneration. Front Neurosci 14: 1–33
- Raccaud M, Friman ET, Alber AB, Agarwal H, Deluz C, Kuhn T, Gebhardt JCM & Suter DM (2019) Mitotic chromosome binding predicts transcription factor properties in interphase. Nat Commun 10: 1–16
- Roussel BD, Kruppa AJ, Miranda E, Crowther DC, Lomas DA & Marciniak SJ (2013) Endoplasmic reticulum dysfunction in neurological disease. Lancet Neurol 12: 105–118
- Tagawa A, Mezzacasa A, Hayer A, Longatti A, Pelkmans L & Helenius A (2005) Assembly and trafficking of caveolar domains in the cell. J Cell Biol 170: 769–779
- von Wichert G (2003) Force-dependent integrin-cytoskeleton linkage formation requires downregulation of focal complex dynamics by Shp2. EMBO J 22: 5023–5035
0 Comments